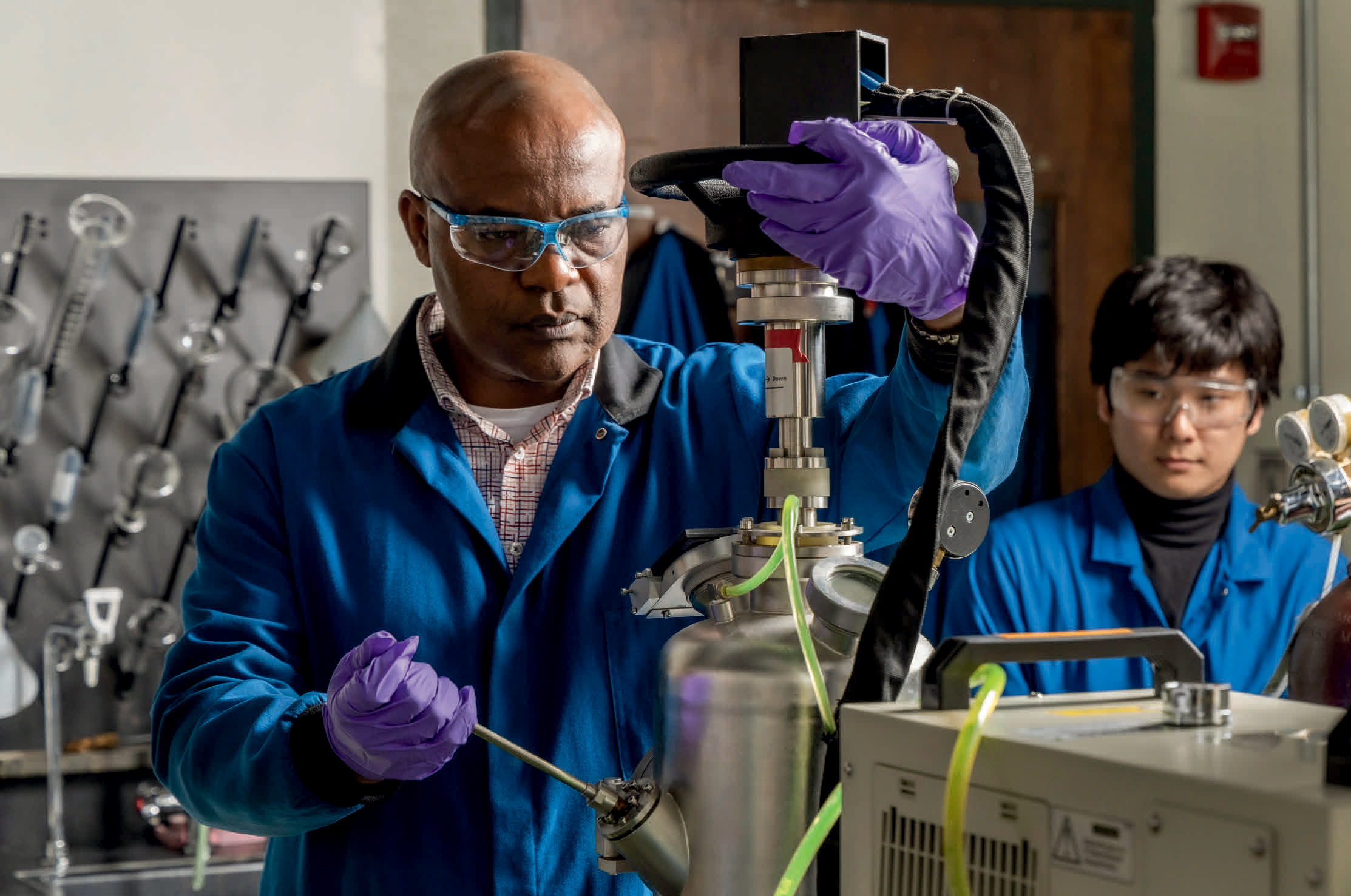
Extending Battery Lifespan and Capacity Through Self-Healing Materials
One of the greatest challenges in the fight against climate change is energy storage. Fossil fuel essentially stores itself, with its energy locked inside its own chemical bonds. But how do you store more sustainable, but otherwise ephemeral, forms of energy, like the power of the wind and sun?
For Eric Detsi, Associate Professor in Materials Science and Engineering (MSE), the answer is batteries, with the caveat that batteries powerful enough to meet the future’s energy demands — the International Energy Agency projects that worldwide battery capacity will need to sextuple by 2030 — do not yet exist.
In most batteries used today, from the disposable alkaline batteries in household appliances like alarm clocks to the rechargeable lithium-ion batteries in hybrid and electric vehicles, the electrodes between which ions flow are typically made of solid materials like metal oxides or graphite. But, as Detsi points out, each cycle of charging and discharging the battery damages the material because the electrodes expand and contract, sometimes by as much as 300%, which is one of the reasons why even rechargeable batteries gradually lose capacity and eventually fail.
“There is a need for materials that can store a large amount of lithium, sodium and magnesium for use in high-performance batteries,” says Detsi. “The problem is that the more lithium, sodium or magnesium a battery material can store, the more it expands and shrinks during charging and discharging, resulting in huge volume change.”
Some researchers, including the late 2019 Nobel laureate John Goodenough, one of the creators of lithium-ion batteries, recently started to develop batteries with liquid electrodes, which don’t break when their volume changes. But liquid electrodes present other challenges, namely the difficulty of safely manufacturing and using batteries that behave like water balloons. In other words, just building larger or liquid batteries won’t work — to design the batteries of the future, researchers will need to create entirely new materials.
What’s more, many of the elements typically used in mass-produced, rechargeable batteries — like lithium and cobalt — are becoming increasingly expensive, not to mention entangled in human rights abuses, as demand for batteries increases. (Last year, Siddharth Kara, a professor at the University of Nottingham, published Cobalt Red: How the Blood of the Congo Powers Our Lives, an exposé about the abysmal labor practices in the Democratic Republic of the Congo, which produces three-quarters of the world’s cobalt.) “The need for high-performance batteries for emerging energy storage applications such as grid-scale storage and electric vehicles led me to study materials for batteries,” says Detsi.
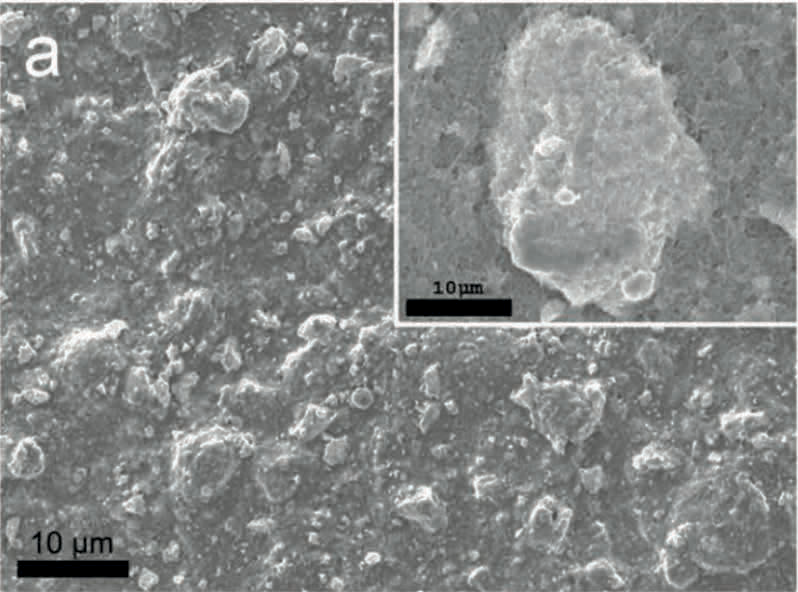
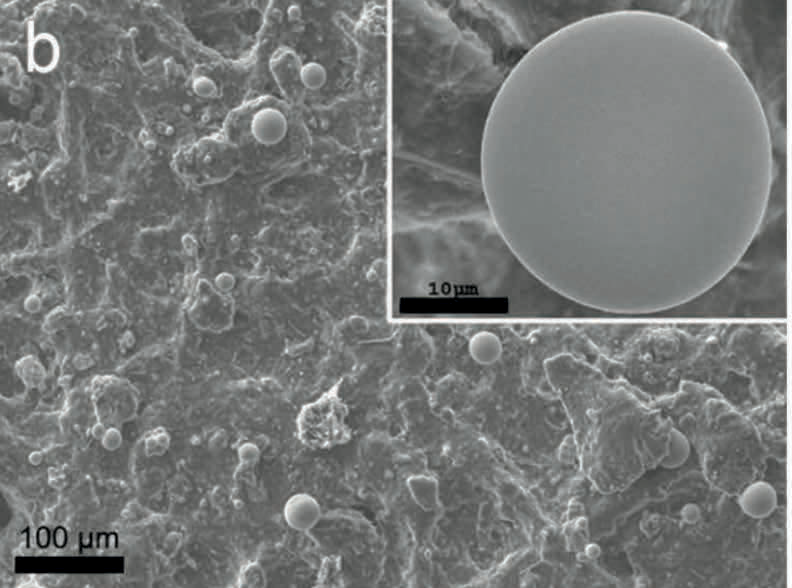
In this microscopic view, a novel battery developed by the Detsi lab using dimagnesium pentagallide (Mg2Ga5), a mixture of magnesium and gallium, is shown before (a) and after (b) the self-healing process takes place.
To that end, his group has been studying batteries made primarily of sodium and magnesium, which are cheaper and less ethically fraught since sodium and magnesium are plentiful in the earth’s crust. More importantly, sodium and magnesium resources are abundant in the U.S. For example, according to the U.S. Geological Survey (USGS), 68.8% of the world’s reserves of sodium carbonate (soda ash) and 14.5% of the world’s sodium chloride (salt), which are needed to make sodium, are found in the U.S.
Detsi’s group is using these metals to develop electrodes that shift between liquid and solid states to avoid damage during charge cycles while still being easy to manufacture. “When the material is in the solid phase, it will start degrading due to the huge volume changes occurring during charge storage,” says Detsi. “However, when the material transforms from solid to liquid, it ‘heals’ itself by recovering from volume-change-induced degradation.”
At first, Detsi demonstrated the feasibility of this approach using an anode — the electrode that collects ions during charging — made of dimagnesium pentagallide (Mg2Ga5), a mixture of magnesium and gallium, the latter of which has a low melting point, making it easy for such alloys to shift from solid to liquid.
In 2019, Detsi’s lab, along with that of Vivek Shenoy, Eduardo D. Glandt President’s Distinguished Professor in MSE, in Mechanical Engineering and Applied Mechanics (MEAM), and in Bioengineering (BE), showed that self-healing anodes made of Mg2Ga5 could withstand more than 1,000 charge cycles. “Before our work,” says Detsi, “the cycle life of state-of-the-art magnesium-ion battery anodes was only 200 cycles.” In other words, the addition of the self-healing anode quintupled the initial lifespan of magnesium-ion batteries.
Earlier this year, Detsi’s lab pushed the envelope even further, using a gallium-indium anode that melts at room temperature, potentially opening the door to commercial applications. The experimental anode survived 2,000 charging cycles while retaining 91% battery capacity. “This is unprecedented,” says Detsi. For context, the iPhone 15 can sustain 1,000 charging cycles while retaining 80% battery capacity.
In order to advance the project, Detsi and his co-authors — Lin Wang and Alexander Ng, recent Ph.D. graduates, and Roxana Family, a postdoctoral fellow — employed a variety of advanced imaging techniques to better understand the material’s transformation from solid to liquid, including X-ray diffraction, X-ray scattering, X-ray spectroscopy and cryogenic scanning electron microscopy. The latter technique involves freezing the liquid metal anodes at different stages to better study the self-healing process, as Detsi and his group described in a 2023 paper published by the American Chemical Society.
Nearly a decade ago, when Detsi and his group started exploring the concept of self-healing sodium- and magnesium-ion batteries, hardly anyone took his ideas seriously. “I remember a reviewer of one of our proposals on sodium-ion batteries asking why sodium-ion batteries are not commercialized if they are so great,” Detsi says. “At the time, there was only one startup company developing sodium-ion batteries. Now there are many across the globe.”
Story by Ian Scheffler, originally published on Penn Engineering Today / Photo by Kevin Monko
Inside Engineering
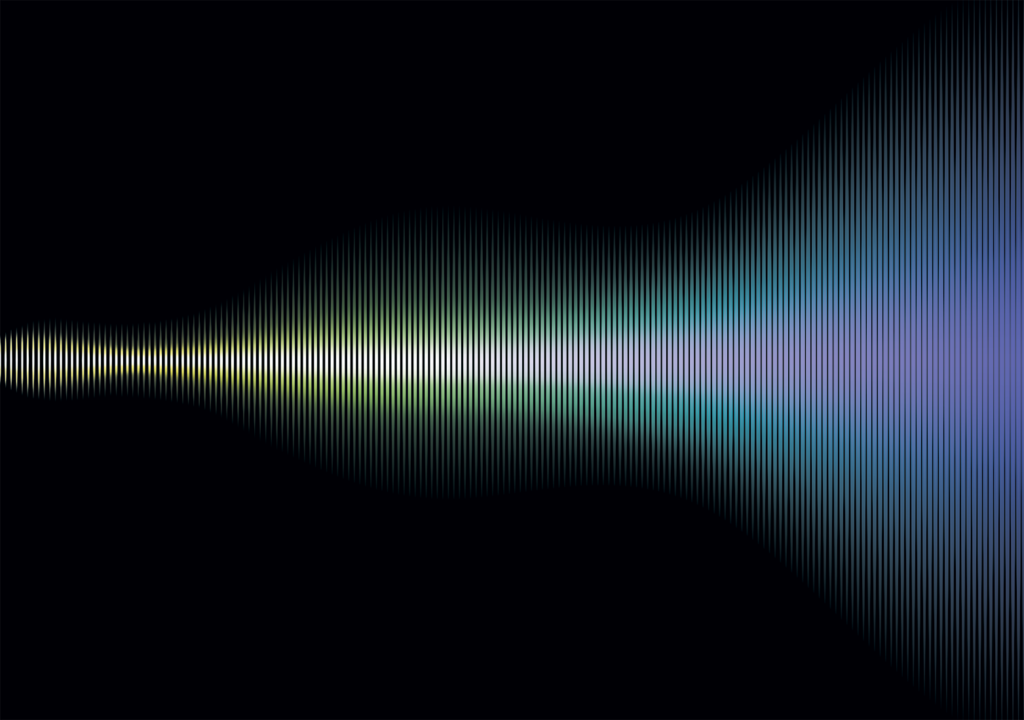
To 6G and Beyond
The electromagnetic spectrum is one of the modern world’s most precious resources. Only a tiny fraction of the spectrum, mostly radio waves representing less than one billionth of one percent of the overall spectrum, is suitable for wireless communication.
It’s a crowded space, with wireless communications mostly using the lower-frequency bands, which are also carefully controlled by the Federal Communications Commission (FCC).
Current wireless networks, including 5G, 4G and 3G, operate from 600 MHz to 6 GHz. Devices use different filters for different frequencies, meaning that the typical smartphone includes upwards of 100 filters to ensure that signals from different bands don’t interfere with one another. The FCC recently made available what is known as the Frequency Range 3 (FR3) band, which includes frequencies from about 7 GHz to 24 GHz, for commercial use. FR3 will likely roll out for 6G or Next G, and current filter technologies in those bands are highly limited.
To combat this issue, Troy Olsson, Associate Professor in Electrical and Systems Engineering (ESE), has collaborated with Mark Allen, Alfred Fitler Moore Professor in ESE, and Firooz Aflatouni, Professor in ESE, to create a new filter that is designed to be adjustable, so that engineers can use it to selectively filter different frequencies rather than have to employ separate filters for each frequency.
This means not having to put another 100+ filters in your phone with many different switches, creating the most viable path to using the FR3 band.
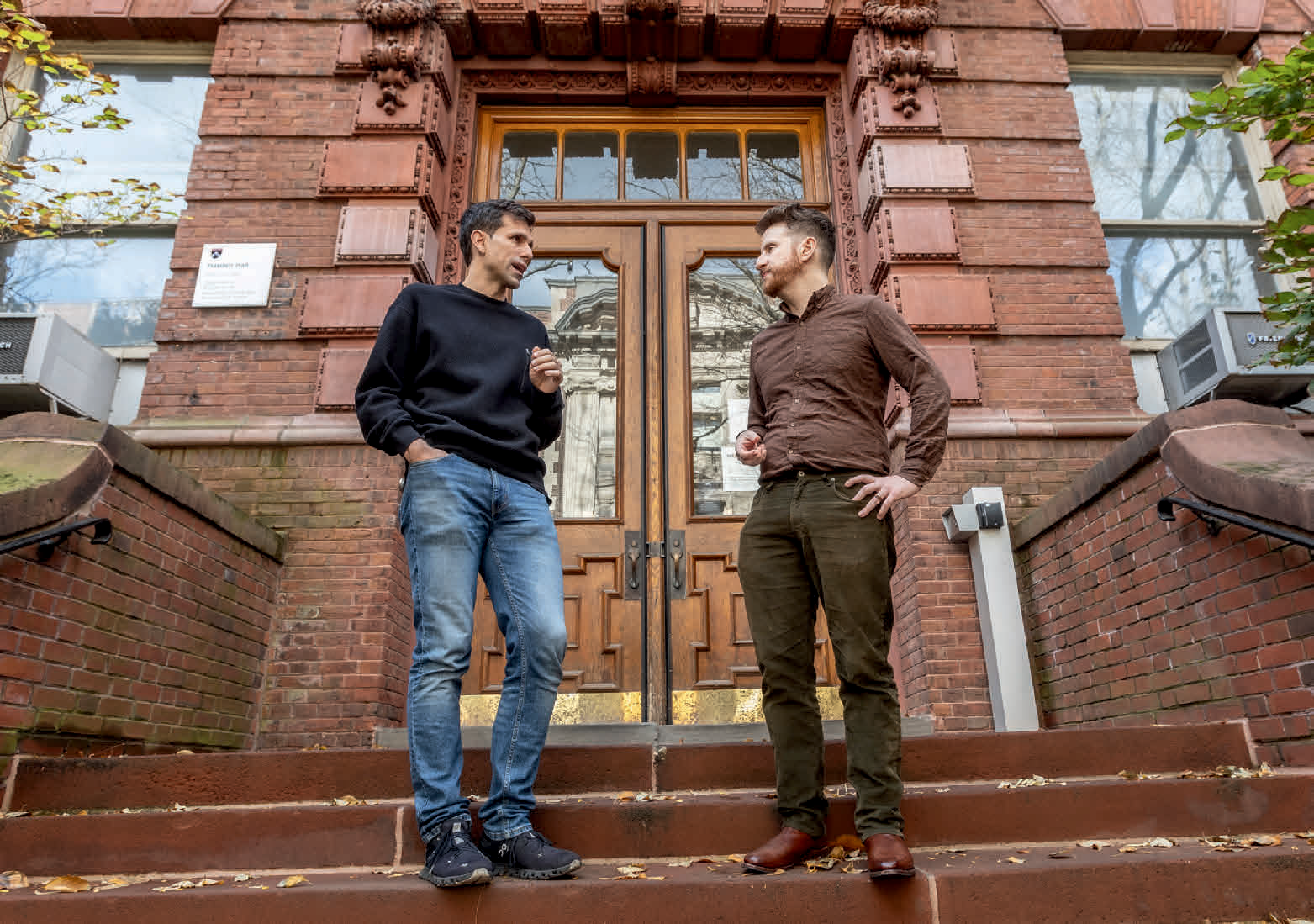
Leveraging AI to Fight Antibiotic Resistance
The rise of drug-resistant bacteria, resulting from years of treatment misuse, is a growing global health crisis that causes approximately 4.95 million deaths per year and threatens to make even common infections deadly.
To combat this problem, education is key (be sure to finish your full course of antibiotics!), but it must be complemented with the development of new antibiotic solutions.
César de la Fuente, Presidential Associate Professor in Psychiatry and Microbiology in Penn Medicine, in Bioengineering and in Chemical and Biomolecular Engineering in Penn Engineering, and in Chemistry in Penn Arts & Sciences, leads a team of interdisciplinary researchers to tackle this looming threat.
First, de la Fuente pioneered an artificial intelligence tool to mine the vast and largely unexplored biological data — more than 10 million molecules — from both modern and extinct organisms to discover new antibiotic candidates. This method dramatically reduces the usual time and costs associated with developing new preclinical drug candidates, and means that new candidates can be identified within hours instead of years.
Now, as de la Fuente works to usher in a new era of antibiotic discovery, he is working with Jacob Gardner, Assistant Professor in Computer and Information Science, whose work explores how
machine learning techniques can be applied to large-scale, high-dimensional optimization problems in the natural sciences.
Together, the team is taking some of the potential antibiotics identified from extinct organisms and using AI to optimize these candidates to be even more effective and broad spectrum.
Departmental Highlights
Bioengineering
Characterizing Cellular Cargo
Jina Ko, Assistant Professor, has created a novel tool to characterize the contents of extracellular vesicles, the cargo-carrying particles excreted by many cells, potentially advancing the early detection of diseases like cancer.
Chemical and Biomolecular Engineering
Liquid Crystal Conveyor Belts
Chinedum Osuji, Eduardo D. Glandt Presidential Professor, has demonstrated that under the right conditions, liquid crystals form astonishing structures reminiscent of biological systems, spontaneously generating filaments and flattened discs that can transport material from one place to another.
Computer and Information Science
Improving Dpression Detection
Sharath Chandra Guntuku, Research Assistant Professor, has collaborated to show that methods used to detect possible depression through language in social media posts break down when applied to posts made by specific racial groups, highlighting the importance of considering the intersection of race, health risks and social media.
Electrical and Systems Engineering
Light-Speed Computing
Nader Engheta, H. Nedwill Ramsey Professor, and Firooz Aflatouni, Professor, have developed a new chip that uses light waves, rather than electricity, to perform some of the complex math essential to training AI, potentially accelerating the processing speed of computers while also reducing their energy consumption.
Materials Science and Engineering
Novel Nanocrystals
For the first time, a team led by Christopher Murray, Richard Perry University Professor, has observed different nanoparticles combining into one superstructure in real time, an advance that could lead to manufacturing materials that combine properties like magnetism and photoluminescence.
Mechanical Engineering and Applied Mechanics
Self-Folding Robots
Cynthia Sung, Associate Professor, has developed CurveQuad, a tiny robot powered by a single motor that uses curved-crease origami to self-fold, unfold, crawl and steer, underscoring origami’s potential in designing low-cost robots. The manufacturing process for CurveQuad is also scalable to large-volume production.